A review of nasal spray formulation excipients and their potential for enhancing drug delivery.
Introduction
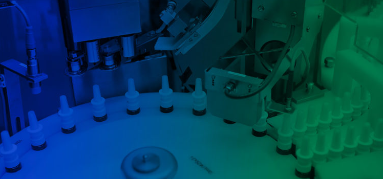
For many years, the nasal route of administration has been used very successfully for the non-invasive delivery of small molecule drugs. The anatomy and physiology of the nasal cavity have been exploited to deliver 1) locally acting medications, to combat ailments such as seasonal and year-round allergies, 2) systemically delivered drugs, via the highly vascularized turbinates (e.g., migraine treatments), and 3) drugs delivered directly to the central nervous system (nose-to-brain delivery), via the olfactory and trigeminal nerves (e.g., seizure treatments) [1].
Some commercially available liquid nasal spray formulations include excipients that can act as penetration enhancers or mucoadhesives, and may thereby provide the benefit of increasing contact/residence time in the nasal cavity or increasing absorption of drug molecules by enhancing permeability through nasal mucosal membranes. As liquid nasal sprays are now being developed to expand their use from traditional small molecule drug delivery systems to delivery of biologics and as a prophylaxis for respiratory diseases (including coronavirus treatments), the inclusion of penetration enhancers and mucoadhesives may become more important. This article, while not intended to be comprehensive, provides information that may assist readers in considering the utility of various penetration enhancers and mucoadhesives for use in research and development.
Rationale for use of penetration enhancers and mucoadhesives
The absorption of drugs through the biological membranes of the nasal cavity is influenced by the molecular weight, size and hydrophilicity/lipophilicity of the drug, as well as the permeability of the membrane itself. Large molecular weight and water-soluble drugs (e.g., proteins and peptides) have relatively low membrane permeability [2]. This, together with the limited residence time within the nasal cavity caused by mucociliary clearance (typically 15-20 minutes) necessitates formulation approaches to increase the contact time of the drug at the site of absorption, and the permeability of the mucosal membrane. One formulation approach that is becoming more widely investigated involves the use of penetration enhancers and/or mucoadhesives.
The year 2020 saw rapid expansion in interest in the use of liquid nasal sprays for the prophylactic treatment of respiratory diseases, fueled by the pharmaceutical industry’s response to the COVID-19 pandemic [3]. These formulations are intended to be retained and act locally within the nasal cavity, the site of first infection. As such, it is important that the residence time of the drug within the nasal cavity is maximized—not to increase time for absorption, but to increase opportunity for the drug to interact with the virus within the nose and prevent it entering deeper into the respiratory system. The use of mucoadhesives in these types of liquid nasal spray formulations may help achieve this objective.
It is important to note that demonstrating the absence of undesirable biological effects is a major barrier to the introduction of new excipients intended to enhance absorption in the nose. For example, some penetration enhancers have been reported to be irritating to the nasal mucosa as well as having a detrimental effect on ciliary activity, thus impacting the integrity of the nasal defense mechanisms. Measuring the ciliary beat frequency of nasal epithelial tissue has been suggested as a tool in the search for safe nasal absorption enhancers [4]. Although mucoadhesives are not normally as irritating to the nasal mucosa as penetration enhancers, they act by increasing the viscosity of the mucus. This, in turn inhibits the effect of ciliary beating, impairing mucociliary clearance [5]. The Inactive Ingredient Database [6] provides information on excipients used in FDA-approved drug products and can be used as a starting point when considering formulation additives.
Penetration enhancers
There are two primary mechanisms for absorption through the nasal mucosa—paracellular transport and transcellular transport [7]. Paracellular transport involves the transient opening of the tight junctions between adjacent cells to enable diffusion through the intercellular space. Transcellular transport involves the disruption of the integrity of the cell membrane lipid bilayer and increased membrane fluidity, thereby reversibly enabling the permeation of drugs. This can result in the opening of the aqueous pores due to calcium ion chelation and/or increase the intracellular delivery using functional moieties [8]. Some surfactants added to improve the physical stability of aqueous nasal suspensions, such as Polysorbate 20 and 80, may also serve as penetration enhancers.
Alkylsaccharides. Alkylsaccharides consist of an aliphatic hydrocarbon chain coupled to a sugar moiety. It has been reported that the alkyl maltosides, including dodecyl maltoside (DDM), enhance transmucosal delivery through both the transcellular and paracellular pathways—temporarily loosening the tight junctions between epithelial cells, as well as increasing the permeability of cell membranes, allowing drug to pass through. For DDM, bioavailability enhancement has been demonstrated from intranasal application of molecules (small molecules, therapeutic proteins, peptides and non-peptide macromolecules) of different molecular weights up to 30 kDa [9].
Bile salts and derivatives. Cholates (e.g., sodium glycocholate, sodium taurocholate) are bile salts that are synthesized from the lipid, cholesterol. Cholesterol is an essential structural component of animal cell membranes, modulating membrane fluidity and functioning in intracellular transport. Due to their structural similarity to cholesterol, it has been postulated that bile salts and their derivatives may cause fluidization of the nasal epithelial cell membranes, thereby increasing transcellular transport [7]. It has been reported that these materials have been shown to effectively promote nasal insulin and peptide drug absorption.
Cyclodextrins. Cyclodextrins are cyclic oligosaccharides produced by the enzymatic degradation of starch. Typical cyclodextrins contain 6 to 8 α-D-glucopyranoside units connected by α(1→4) linkages in a ring, creating a cone shape. There are three native cyclodextrins: α-cyclodextrin (a six sugar-ring molecule), β-cyclodextrin (a seven sugar-ring molecule), and γ-cyclodextrin (an eight sugar-ring molecule) enclosing a cavity of about 6, 8 and 10 Å, respectively [10, 11]. These molecules have a hydrophilic outer surface and a relatively hydrophobic inner cavity that allows entrapment of a wide range of drug molecules, including proteins and peptides. These inclusion complexes are formed by weak non-ionic interactions (e.g., van der Waals forces), hydrophobic bonding and hydrogen bonding between the cyclodextrin cavity and the drug molecule [12, 13]. Cyclodextrins can increase drug solubility at the absorption site and act as permeation enhancers by carrying the drug from the bulk solution to the lipophilic surface of biological membranes, where the drug molecules partition into the lipophilic membrane. Cyclodextrin/chitosan nanoparticles have been used to combine the solubilizing properties of anionic cyclodextrins and the mucoadhesive properties of cationic chitosan to prolong the residence time on, and the permeation through, the mucosal membrane [10].
Cyclopentadecanolide. Cyclopentadecanolide is a natural macrolide lactone that is produced synthetically by ring expansion of cyclotetradecanone, or the depolymerization of polyesters of 15-hydroxypentadecanoic acid. Macrolides consist of a large macrocyclic lactone ring, usually 14- to 16-membered. Cyclopentadecanolide is a hydrophobic molecule that can interact with the hydrophobic chains of lipids within the cell membranes and has been shown to enhance the absorption of drugs through the nasal mucosa [14].
Diethylene glycol monoethyl ether. Diethylene glycol monoethyl ether is a hydroalcoholic solvent that has a long history of use as a solvent and penetration enhancer in various pharmaceutical dosage forms, and its use in liquid nasal spray formulations has increased in recent years. Although its mechanism of action is not fully understood, in the case of the stratum corneum, it appears to interact strongly with the water of the intercellular path, facilitating the movement of excipients and/or active pharmaceutical ingredients (APIs)via the intercellular route [15].
Fatty acids. Fatty acids are carboxylic acids with either a saturated or unsaturated long aliphatic chain (e.g., oleic acid, capric acid). Most naturally occurring fatty acids have an unbranched chain of an even number of carbon atoms—between 4 and 28 atoms. Fatty acids have shown a tight junction modulatory effect and enhanced permeation of drugs through the epithelial cell barrier. It has been suggested that they may alter membrane permeability by increasing the fluidity of the membrane, or through Ca2+ dependent tight junction mechanisms. One suggested mechanism of penetration enhancement for a medium chain fatty acid, capric acid, is by activation of phospholipase-C and an increase of intracellular calcium levels, resulting in contraction of actin microfilaments and dilation of the tight junctions [16].
Phospholipids. Phospholipids are a class of molecule that has a hydrophilic “head” containing a negatively charged phosphate group, and two hydrophobic “tails” derived from fatty acids, joined by a glycerol molecule. They are a key component of all cell membranes and can form lipid bilayers because of their amphiphilic nature—the tail regions, being repelled by water and slightly attracted to each other, congregate together, exposing the head regions to the exterior. Liposomes are biodegradable and biocompatible vesicles composed of one or more phospholipid bilayers surrounding an inner aqueous compartment. This structure enables liposomes to encapsulate drugs of different size and lipophilicity. Hydrophilic drugs are encapsulated into the aqueous compartment, lipophilic drugs are contained inside the bilayer, and amphiphilic drugs partition themselves between these two regions. The similarity between the lipid composition of liposomes and cell membranes enables liposomes to be used as physiologically acceptable drug delivery systems [17]. One example of a phospholipid is phosphatidylcholine, which is the major component of lecithin.
Surfactants. Surfactants are compounds that are commonly used to lower the surface tension (or interfacial tension) between two liquids, for example, when formulating and stabilizing emulsions. They are amphiphilic molecules, containing both hydrophilic and hydrophobic groups. Surfactants can be used as very effective permeation enhancers, increasing the fluidity of cell membrane lipids. However, epithelial toxicity, ciliostatic activity and nasal irritation are some drawbacks [18]. Non-ionic surfactants are generally less irritating and better tolerated than anionic and cationic surfactants. Examples of non-ionic surfactants that have been used in intranasal drug delivery systems are Poloxamer 188, Polysorbate 20 and Polysorbate 80.
Thiomers. Thiomers enhance the permeability of drugs via the reversible opening of the tight junctions between epithelial cells. They have the potential benefit of not being absorbed through the nasal mucosa, as can happen with lower molecular weight enhancers. (For more information on thiomers, see the section on mucoadhesives.)
Other delivery systems. In addition to penetration enhancers, other delivery systems have been investigated to increase the efficiency of drug delivery—including cell-penetrating peptides, enzyme inhibitors (to reduce drug inactivation by mucosal proteases and reductases), polymeric nanoparticle carriers, microemulsions and nanoemulsions.
Mucoadhesives
The nasal cavity is lined with a layer of mucus and hairs (cilia) that trap inhaled particles and pathogens. The cilia are in a constant wavelike movement, sweeping the mucus and entrapped particles towards the pharynx for ingestion and clearance by the gastrointestinal tract [2, 5, 7]. This process of mucociliary clearance limits the residence time of particles within the nasal cavity to 15-20 minutes. Mucoadhesives are used in liquid nasal spray formulations to increase the viscosity of the mucus. This, in turn, inhibits the effect of ciliary beating, impairing mucociliary clearance, and increasing the residence time of the drug on the nasal mucosa to allow more time for passive diffusion. Additionally, mucoadhesives can provide sustained drug release and reduce the degradation of vulnerable, small molecular weight and peptide-based drugs [19].
The inclusion of mucoadhesives can have the effect of increasing the viscosity of a formulation, leading to a narrower spray plume and larger spray droplets [20-22]. As with penetration enhancers, mucoadhesives may also play other roles in a formulation. For example, due to their viscosity-enhancing properties and thixotropic nature, they may act as suspending and stabilizing agents. Additionally, by increasing the droplet size of the spray, the increased formulation viscosity may play a part in controlling where, within the nasal cavity, spray droplets would be deposited and reduce the possibility of unintended lung delivery [23].
Cellulose and derivatives. Cellulose is a polysaccharide consisting of a linear chain of several hundred to more than 10,000 β(1→4) linked D-glucose units. Cellulose derivatives used as mucoadhesives include carboxymethyl cellulose (CMC), hydroxypropyl cellulose (HPC) and hydroxypropyl methylcellulose (HPMC) [24]. CMC, specifically the sodium salt (NaCMC) is an extensively used mucoadhesive polymer. It is an anionic polymer that generates more hydrogen bonding than non-ionic polymers, thereby possessing better mucoadhesion. HPMC is used not only for mucoadhesion but also as a controlled release mechanism. It is a non-ionic polymer lacking a proton-donating carboxylic group, which causes lesser hydrogen bonding than CMC. HPMC exhibits thermal gelation, increasing the viscosity as the temperature is raised. HPC, like HPMC, is a non-ionic, water-soluble, cellulose ether with the thickening and stabilizing properties characteristic of other water-soluble cellulose polymers. While HPC and HPMC are insensitive to electrolytes, CMC is affected both by ionic strength and pH.
Chitosan and derivatives. Chitosan is a cationic polysaccharide comprised of glucosamine and N-acetyl glucosamine copolymers linked by β(1→4) glycosidic bonds, and is obtained by the partial deacetylation of chitin [19]. Chitosan polymers vary in their degree of deacetylation, molecular weight (50 kDa to 2,000 kDa), and viscosity [25]. Chitosan has one primary amino and two free hydroxy groups for each C6 building unit [26], giving the capability of both hydrogen bonding and covalent bonding. The high charge density of the amino groups enables chitosan to chemically react with anionic systems, giving rise to a strong electrostatic interaction with the negatively charged mucosal glycoproteins. Microspheres can be prepared by reacting chitosan with controlled amounts of multivalent anions, resulting in intermolecular cross-linking. These microspheres can provide controlled release of drugs, improve the bioavailability of degradable substances (such as proteins) and improve the uptake of hydrophilic substances across the epithelial layers. Unfortunately, the solubility and mucoadhesive nature of chitosan requires an acidic pH (pH < 6), which can limit its use, as many biologics are not stable at low pH. As a result, various chitosan derivatives have also been investigated.
Gums. Other naturally occurring polysaccharides have also been shown to exhibit mucoadhesive properties [24], including alginates, guar gum, pectin and xanthan gum.
Alginates are salts of alginic acid, an anionic polymer obtained from brown seaweed. Alginic acid is a linear copolymer, comprising blocks of (1→4)-linked β-D-mannuronate and α-L-guluronate residues covalently linked in different sequences. They form viscous gums and have good mucoadhesive properties due to the presence of the carboxylic acid moiety, which causes hydrogen bonding with the glycoprotein of mucin.
Guar gum is a non-ionic polysaccharide based on galactose and mannose units and is extracted from guar beans. It consists of a linear chain backbone of β(1→4)-linked mannose units to which galactose units are (1→6)-linked at every second mannose, forming short side-branches. The mucoadhesive properties of guar gum are due to hydrogen bonding with the mucin glycoproteins. Additionally, there is a synergistic rheological interaction between guar gum and xanthan gum—the viscosity of the gum combination is higher than the sum of the single polymer solutions.
Pectin is a natural heterogenous anionic polysaccharide that is extracted from citrus peel or apple pomace. It contains linear chains of (1→4)-linked α-D-galacturonic acid residues that contain carboxyl groups. The mucoadhesive properties of pectin come via the formation of hydrogen bonds between the carboxylic acid groups and mucin, and electrostatic interaction between pectin and the mucin molecule. Mucin and pectin are both negatively charged; therefore, increasing the concentration of pectin in an aqueous medium increases electrostatic repulsion with mucin. This repulsion causes uncoiling of the polymer chain, facilitating interpenetration, entanglement and adhesion.
Xanthan gum is an anionic polysaccharide produced by the fermentation of glucose and sucrose. The xanthan molecule consists of a backbone of β(1→4)-linked D-glucose residues. Trisaccharide side chains containing two mannose units separated by guluronic acid are linked to every other glucose unit. Although xanthan gum has negatively charged carboxyl groups on the side chains, the steric hindrance of these side chains inhibits access to the charged mucin groups, leading to a low level of mucoadhesion [27].
Lectins. Lectins are carbohydrate-binding proteins that are capable of binding to specific sugar moieties [28, 29]. Due to their ability to cross-link sugar-containing macromolecules, they are able to coagulate these molecules, forming a thickened mass (agglutination). Certain lectins can bind to the mucosa, and then either stay on the cell surface or become internalized by endocytosis. As such, lectins not only act as mucoadhesives, but can aid in delivering drug into cells (active cell-mediated drug uptake). Wheat germ agglutinin (WGA) is a lectin that specifically binds to N-acetyl-D-glucosamine and sialic acid moieties and exists mostly as a heterodimer of 38,000 Da. Both N-acetyl-D-glucosamine and sialic acid are found in the nasal cavity, especially the olfactory mucosa. One of the benefits of WGA is its low potential for causing immunogenic reactions compared to other lectins, which may be powerful toxins (e.g., ricin).
Poloxamer. Poloxomers are non-ionic, difunctional, triblock copolymers composed of a central hydrophobic chain of polyoxypropylene between two hydrophilic chains of polyoxyethylene. Poloxomer gels are thermosensitive, exhibiting phase transitions from liquids (sols) to mucoadhesive gels upon exposure to the nasal temperature. The actual temperature of gelation can be adjusted by the addition of other excipients such as polycarbophil, polyethylene glycol and benzalkonium chloride. One poloxamer of interest for nasal delivery, due to its low toxicity, high solubility and bioadhesive characteristics, is Poloxamer 407. Their comparatively low molecular weight and non-ionic nature makes poloxamers relatively weak mucoadhesive agents. To enhance their mucoadhesion and drug retention, a low concentration (0.2-0.5%) of other mucoadhesive polymers, e.g., chitosan, sodium carboxymethyl cellulose, hydroxypropyl methylcellulose or hydroxypropyl cellulose can be added to the poloxamer gel [24,28].
Polyacrylic acid. Polyacrylic acid is a synthetic, high-molecular weight polymer of acrylic acid. Mucoadhesion occurs through hydrogen bonding between the carboxylic acid functionality of the mucoadhesive polyacrylic acid and the sialic acid/carboxylic acid groups of the glycoprotein component of mucus [28, 30-31]. Although these types of bonding forces are weak, numerous interaction sites lead to strong mucoadhesion.
Polyethylene glycol. Polyethylene glycols (PEGs) are hydrophilic, water-soluble, polyether compounds. PEGs are prepared by polymerization of ethylene oxide and are commercially available over a wide range of molecular weights. Polymer chain length plays an important role in mucoadhesion, with longer chain lengths leading to an increase in mucoadhesive properties. Having flexible polymer chains helps penetration and entanglement with the mucosal layer, thereby improving mucoadhesion [32]. In the case of PEG, a polymer of 20 kDa shows negligible mucoadhesive properties while one of 200 kDa has been shown to exhibit improved mucoadhesion and one of 400 kDa has excellent mucoadhesion [33].
Thiomers. Thiols are organosulfur compounds of the form R−SH, where the –SH functional group is referred to as either a thiol group, a sulfhydryl group or a sulfanyl group. Thiomers, or thiolated polymers, are polymers that have been modified by the addition of thiol-bearing side chains. These thiol groups can form covalent, disulfide bonds with the cysteine-rich subdomains of mucus glycoproteins that make up the mucus gel layer, via thiol/disulfide exchange reactions and/or a simple oxidation process. As a result, thiomers mimic naturally secreted mucus glycoproteins and can improve mucoadhesion by up to one hundred times [28, 29, 34]. Examples are thiomers based on chitosan, hyaluronic acid, gelatin, polyacrylates and cyclodextrins.
In summary
Liquid nasal sprays continue to be a very useful, patient-friendly, non-invasive drug delivery mechanism. Different formulation approaches, including a greater emphasis on the use of penetration enhancers and/or mucoadhesives, are being investigated to facilitate their evolution from being a delivery system for small molecules to one that may be better able to deliver biologics and prophylactics.
References
1. Ehrick JD, Shah SA, Shaw C, Kulkarni VS, Coowanitwong I, De S, Suman JD. Book chapter. “Considerations for the development of nasal dosage forms” in “Sterile product development: Formulation, process, quality and regulatory considerations.” AAPS Advances in the Pharmaceutical Sciences Series. Springer, New York, NY. 6: 99-144 (2013).
2. Thorat S. Formulation and product development of nasal spray: An overview. Sch. J. App. Med. Sci. 2016; 4(8D): 2976-2985.
3. Go CC, Pandav K, Somagutta MR, Go JK, Bethencourt-Mirabal A, Bhatt K, Sanchez-Gonzalez MA, Ferrer G. Intranasal therapy and COVID-19: A comprehensive literature review. J. Allergy Infect. Dis. 2021; 2(1): 9-16.
4. Merkus FWHM, Schipper NGM, Hermens WAJJ, Romeijn SG, Verhoef JC. Absorption enhancers in nasal drug delivery: Efficacy and safety. J. Controlled Release. 1993; 24 (1-3): 201-208.
5. Bourganis V, Kammona O, Alexopoulos A, Kiparissides C. Recent advances in carrier mediated nose-to-brain delivery of pharmaceutics. Eur. J. Pharm. Biopharm. 2018; 128: 337-362.
6. United States Food and Drug Administration. Inactive Ingredients Database Download. www.fda.gov/drugs/drug-approvals-and-databases/inactive-ingredients-database-download.
7. Maggio ET. Absorption enhancing excipients in systemic nasal drug delivery. J. Excipients and Food Chem. 2014; 5 (2): 100-112.
8. Ghadiri M, Young PM, Traini D. Strategies to enhance drug absorption via nasal and pulmonary routes. Pharmaceutics. 2019; 11: 113.
9. Maggio ET, Pillion DJ. High efficiency intranasal drug delivery using Intravail® alkylsaccharide absorption enhancers. Drug Deliv. and Transl. Res. 2013; 3: 16-25.
10. Muankaew C, Loftsson T. Cyclodextrin-based formulations: a non-invasive platform for targeted drug delivery. Basic Clin. Pharmacol. Toxicol. 2017; 122 (1): 46-55.
11. Vikas Y, Sandeep K, Braham D, Manjusha C, Budhwar V. Cyclodextrin complexes: An approach to improve the physicochemical properties of drugs and applications of cyclodextrin complexes. Asian J. Pharm. 2018; 12 (2): 394-409.
12. Bender ML, Komiyama M. Cyclodextrin chemistry. Springer, Berlin. (1978).
13. Connors KA. The stability of cyclodextrin complexes in solution. Chem. Rev. 1997; 97: 1325-1358.
14. Li, Z. “Study of the enhancement effect of cyclopentadecanolide on protein permeation through lipid membranes.” (2004). Doctoral Dissertations. 229. University of New Hampshire.
15. Osborne DW, Musakhanian J. Skin penetration and permeation properties of Transcutol®—Neat or diluted mixtures. AAPS PharmSciTech. 2018; 19 (8): 3512-3533.
16. Del Vecchio G, Tscheik C, Tenz K, Helms HC, Winkler L, Blasig R, Blasig IE. Sodium caprate transiently opens claudin-5-containing barriers at tight junctions of epithelial and endothelial cells. Mol. Pharm. 2012; 9: 2523–2533.
17. Cagdas M, Sezer AD, Bucak S. Book chapter. “Liposomes as potential drug carrier systems for drug delivery” in “Application of nanotechnology in drug delivery”. Ed. Sezer AD; Publ: IntechOpen (2014).
18. Jassim ZE, Al-Akkam EJ. A review on strategies for improving nasal drug delivery systems. Drug Invent. Today. 2018; 10: 2857-2864.
19. M Ways TM, Lau WM, Khutoryanskiy VV. Chitosan and its derivatives for application in mucoadhesive drug delivery systems. Polymers (Basel). 2018; 10(3): 267.
20. Dayal P, Shaik MS, Singh M. Evaluation of different parameters that affect droplet size distribution from nasal sprays using the Malvern SprayTec®. J. Pharm. Sci. 2004; 93: 1725-1742.
21. Kulkarni V, Brunotte J, Smith M, Sorgi F. Investigating influences of various excipients of the nasal spray formulations on droplet size and spray pattern. Poster at AAPS Annual Meeting (2008).
22. Kulkarni VS, Shaw C, Smith M, Brunotte J. Characterization of plumes of nasal spray formulations containing mucoadhesive agents sprayed from different types of devices. Poster at AAPS Annual Meeting (2013).
23. Pu Y, Goodey AP, Fang X, Jacob K. A comparison of the deposition patterns of different nasal spray formulations using a nasal cast. Aerosol Sci. Technol. 2014; 48(9): 930-938.
24. Anil A, Sudheer P. Mucoadhesive polymers: A review. J. Pharm. Res. 2018; 17(1): 47-55.
25. Singla AK, Chawla M. Chitosan: Some pharmaceutical and biological aspects—An update. JPP 2001; 53: 1047-1067.
26. Dhawan S, Singla AK, Sinha VR. Evaluation of mucoadhesive properties of chitosan microspheres prepared by different methods. AAPS PharmSciTech. 2004; 5(4): Article 67.
27. Harding SE. Mucoadhesive interactions. Biochem. Soc. Trans. 2003; 31(5): 1036-1042.
28. Anand U, Feridooni T, Agu RU. Book chapter. “Novel mucoadhesive polymers for nasal drug delivery” in “Recent advances in novel drug carrier systems.” Ed. Sezer AD; Publ: InTech. 11: 315-330 (2012).
29. Mythri G, Kavitha K, Kumar MR, Jagadeesh Singh SD. Novel mucoadhesive polymers –A review. J. Appl. Pharm. Sci. 2011; 01(08): 37-42.
30. Park H, Robinson JR. Mechanisms of mucoadhesion of poly(acrylic acid) hydrogels. Pharm. Res. 1987; 4(6): 457-64.
31. Patel MM, Smart JD, Nevell TG, Ewen RJ, Eaton PJ, Tsibouklis J. Mucin/poly(acrylic acid) interactions: A spectroscopic investigation of mucoadhesion. Biomacromolecules. 2003; 4(5): 1184-1190.
32. Roy S, Pal K, Anis A, Pramanik K, Prabhakar B. Des. Polymers in mucoadhesive drug-delivery systems: A brief note. Monomers Polym. 2009; 12: 483-495.
33. Middleton DL, Leung SS, Robinson JR. In “Bioadhesive drug delivery systems.” Ed. Lenaerts V, Gurny R; CRC Press, Boca Raton, FL. 189 (1990).
34. Bernkop-Schnurch A. Thiomers: a new generation of mucoadhesive polymers. Adv. Drug Deliv. Rev. 2005; 57(11): 1569-1582.